Sustainable Management of Medicago sativa for Future Climates: Insect Pests, Endophytes and Multitrophic Interactions in a Complex Environment
- 1AgResearch Ltd., Lincoln, New Zealand
- 2State Key Laboratory for Biology of Plant Diseases and Insect Pests, Institute of Plant Protection, Chinese Academy of Agricultural Sciences, Beijing, China
- 3AgResearch Ltd., Grasslands Research Centre, Palmerston North, New Zealand
- 4Riddet Institute, Massey University, Palmerston North, New Zealand
Medicago sativa L. (alfalfa, syn. lucerne) is an important forage crop for livestock, which is subject to attack from a range of insect pests and susceptible to diseases that can reduce production and persistence. This review considers the main insect pests affecting M. sativa in China and New Zealand as well as the wider plant resistance mechanisms and multitrophic interaction that occur between plants, insect pests, entomopathogens, endophytes, the environment, and climate change. This is with a view to identifying new research opportunities applicable to M. sativa that can be applied to improving production and persistence of this important agricultural crop. These opportunities include identification and activity of entomopathogens/endophytes (e.g., Bacillus and Pseudomonas spp., Metarhizium spp.) and plant growth enhancers (Trichoderma), as well as multitrophic plant-insect-microbial interactions.
Introduction
Medicago sativa L. (alfalfa, syn. lucerne) is an important perennial leguminous forage worldwide (Michaud et al., 1988; Huyghe, 2003; Lamb et al., 2006; Veronesi et al., 2010; Annicchiarico et al., 2015). The widespread use of M. sativa is due to its high adaptability for growth in a range of environments, particularly under drought conditions (Annicchiarico, 2007; Huang et al., 2018), its ability to form symbiosis with rhizobium and biologically fix nitrogen (Carlsson and Huss-Danell, 2003), its high protein value (Vance et al., 1979; Ruckle et al., 2017), and forage yield potential, either under grazing or “cut and carry” cropping regimens (Small, 1996). It can be grown with both temperate and tropical grasses, or as a standalone crop (Capstaff and Miller, 2018), across a range of climates. Nitrogen fixation by rhizobia associated with roots not only provides substantial amounts of nitrogen to plants and soil, but reduces the need for artificial nitrogen fertilizers (Carlsson and Huss-Danell, 2003). In many cases, there is a positive correlation between nitrogen fixation and legume dry matter yields (Carlsson and Huss-Danell, 2003).
In November 2019, a bilateral workshop between AgResearch (New Zealand) and the Institute of Plant Protection-China Academy of Agricultural Science (IPP-CAAS, China), was held to discuss opportunities to cooperatively address challenges in agricultural production common to both countries. Critical challenges to agriculture and M. sativa in particular, were the growing impact of abiotic and biotic threats on insect pests, biocontrol, beneficial microbes (e.g. entomopathogens, soil microbes), abiotic environmental stresses on M. sativa, and the current and future state of knowledge around these aspects. This review examines how these aspects influence plant growth, persistence, and quality in general with a focus on M. sativa where appropriate. In particular, the trophic interactions, that have been observed in M. sativa and other plants species are discussed with a view of establishing areas for future research.
In China, M. sativa is grown for livestock and poultry, with the area sown continuing to expand and demand higher than current supply. The plant is considered integral to the transformation of traditional agriculture (Zhang et al., 2018; Xu et al., 2021). It also provides an important alternative to overgrazing of ecologically sensitive grassland environments (Zhang et al., 2018; McNeill et al., 2021). Medicago sativa is seen as an important crop in the development of animal husbandry, increasing farmers' income, and promoting social and economic development in rural areas (Li, 2019; Xu et al., 2021). The most recently available data show that in 2017, 4.15 million ha2 of alfalfa was being grown with a total yield of 29.3 M tons (National Animal Husbandry Service, 2017). In New Zealand, M. sativa has been promoted as a suitable forage species for New Zealand dryland systems for over 100 years (Moot, 2012). It is considered an important dryland species for grazing and stored winter forage and is particularly valuable to farmers in environments where traditional ryegrass-white clover plant species cannot persist (Avery et al., 2008; Moot, 2012). It is also grown under irrigation as a forage grazing crop for dairy cows (Smith, 2015). Medicago sativa in New Zealand is planted either as a monoculture, destined to be harvested for stored winter feed and, to a lesser extent, for feed pellet production, or when utilized for grazing, either as a monoculture or in combination with a grass species, often tall fescue, Festuca arundinacea Schreb. (Poales: Poaceae). The plant is grown across 200,000 ha producing approximately 2.4 M tons annually and additionally fixes 30 kg N2/ton of legume grown. Animal production from M. sativa yields approximately 700 kg of red meat per ha (D. Moot, Lincoln University, pers. communication). Primary production areas outside China and New Zealand include the United States, Canada, Italy, France, south Russia, Argentina, Chile, South Africa and Australia (Yuegao et al., 2009).
Major Insect Pests of M. Sativa
Worldwide, a range of insect pests (and plant parasitic nematodes and diseases) are known to attack M. sativa, resulting in yield reduction, loss of persistence of stands and plant quality (Leath et al., 1988; Manglitz and Ratcliffe, 1988; Compendium of Alfalfa Diseases Pests, 2016). Losses occur both above- and below- ground and are affected directly through feeding on the foliage or roots, as the result of insect-mediated virus transmission (e.g., aphids) or the ingress of pathogens through wounds caused by insects (Godfrey et al., 1986). The main pests in China are aphids and thrips, followed by alfalfa weevil [Hypera postica (Gyllenhal) (Coleoptera: Curculionidae)]. Aphids and thrips are the most widespread taxa throughout the regions where M. sativa is grown, while H. postica is a significant pest in the Ningxia and Xinjiang regions. Other pests include Heliothis viriplaca (Hufnagel) (= Heliothis dipsacea) (Lepidoptera: Noctuidae) (He et al., 1997) and the beet webworm (Loxostege spp. L.) (Zhang et al., 2005a). Several species of aphids are damaging to M. sativa, with Acyrthosiphon kondoi (Shinji et Kondo), Aphis craccivora (Koch), Acyrthosiphon pisum (Harris) and Therioaphis trifolii (Monell) being the main pest species. Similarly, several species of thrips attack the crop with the major pest being Odontothrips loti (Haliday). Thrips tabaci Lindeman, Frankliniella occidentalis (Perg.) and Frankliniella intonsa (Trybom) also cause damage. Aphids and thrips reduce both the yield and nutritional value of M. sativa (Zhang et al., 2005b, 2017; Wu et al., 2013) and act as vectors of viral plant diseases. Aphids (Garran and Gibbs, 1982; Roumagnac et al., 2015) and thrips (Li J. et al., 2021) have been reported to be carriers of alfalfa mosaic virus and alfalfa leaf curl virus. As the area of M. sativa cultivation increases, so too does the incidence and impact of insect pests and viruses (Wang et al., 2021), seriously restricting further development of the industry. Based on 2017 data, it was estimated that M. sativa pests cause at least 20% yield loss, with an average direct economic loss of 9.144B ¥ p.a. (2.03B NZD p.a.; 1.44B USD) (Li et al., 2020).
In New Zealand, insect pests are a persistent and significant economic cost to grassland and forage production systems (Zydenbos et al., 2011; Jackson et al., 2012; Ferguson et al., 2019), with a few key pests having a major impact on production and longevity of M. sativa. These key species are all exotic (i.e., non-native to New Zealand) and comprise Sitona discoideus Gyllenhal, three aphid species (spotted alfalfa aphid (Therioaphis maculata (Buckton), blue green aphid (Acyrthosiphon kondoi Shinji), and A. pisum. Lesser pests are white fringed weevil [Naupactus leucoloma (Boheman)] and little fringed weevil [Atrichonotus taeniatulus (Berg)]. Like many Sitona species, Sitona discoideus causes both above-ground damage from adult feeding on foliage, and below ground damage to firstly the nitrogen-fixing root nodules and subsequently the root system (Goldson et al., 1988), resulting in both short- and long-term reduction in yields (Goldson et al., 1985; Goldson and Muscroft-Taylor, 1988). Prior to the introduction of biological control agents, high populations of aphids caused death of seedlings and significant yield losses in established stands (Kain and Trought, 1982; Cameron et al., 1983). The introduction and establishment of parasitoid biological control agents as part of classical biocontrol programmes was effective in controlling S. discoideus (Goldson et al., 1990) and the three aphid species (Cameron and Walker, 1989), along with the releases of aphid resistant cultivars (Kain and Trought, 1982).
Endophytes
Bacterial Endophytes
Bacteria belonging to the ‘root-colonizing rhizosphere-competent bacteria’ including members of the genera Pseudomonas (e.g. P. fluorescens), Azospirillum (e.g. A. brasilense), and Bacillus are often also found as colonizers of the internal tissue of plants (Hardoim et al., 2008). Root nodules of leguminous plants have been found to host large population of endophytic bacteria of diverse genera and species which are unrelated to rhizobial symbiotic nitrogen fixing bacteria (Zakhia et al., 2004; Muresu et al., 2008). Stajković et al. (2009) found that co-inoculation of non-rhizobial strains with Sinorhizobium meliloti positively influenced M. sativa nodule numbers, but significant effects on growth parameters with respect to inoculation with S. meliloti alone were absent. However, single inoculation with other non-rhizobial strains caused significant increase in shoot and root parameters compared to uninoculated plants, indicating that non-rhizobial strains possess plant growth promoting potential.
Research in China has identified endophytic fungi (Rhizoctonia solani, Trichoderma harzianum and T. atrobrunneum) and bacterial endophytes (Bacillus and Pseudomonas species) in M. sativa. The bacterial endophytes have been found to be active against the root rot pathogen Fusarium oxysporum F. sp. Medicaginis (Chen et al., 2020). However, there is a paucity of research to demonstrate whether these fungi have other benefits to M. sativa. Conversely, endophyte metabolites may also benefit plant pests. For example, the presence of a Bacillus strain isolated from M. sativa seed was shown to improve the longevity of the free-living nematode Caenorhabditis elegans (Maupas) (Zhang et al., 2018).
Knowledge of bacterial endophytes with demonstrated insecticidal effects is much less developed. Perhaps the most prominent example is Bacillus thuringensis (Lacey et al., 2015), one of the few bacterial endophytes deployed as a commercial biocontrol agent against Lepidoptera. Another example is Brevibacillus laterosporus (Laubach) Shida et al., 1996, originally isolated from surface-sterilized cabbage leaves (Ormskirk et al., 2019). Two Bacillus laterosporus Laubach strains have been shown to exhibit pathogenicity against selected Lepidoptera, Coleoptera, Diptera, and nematodes, as well as antimicrobial activity especially against bacteria and fungi (De Oliveira et al., 2004; Ruiu, 2013). Of particular interest are two strains isolated in New Zealand that cause mortality against larvae of the diamondback moth (Plutella xylostella L.) (van Zijll de Jong et al., 2016). Although B. laterosporus has not yet been found in M. sativa, other endophytic bacteria belonging to the genus Brevibacillus were isolated and exhibited a positive effect on the number of M. sativa nodules while increasing shoot and root parameters (Stajković et al., 2009).
Fungal Endophytes
Fungal entomopathogens (Beauveria spp.), often solely considered as entomopathogens, have been shown to play additional roles in controlling plant pathogens, plant growth promotors and beneficial rhizosphere colonizers (Jaber and Ownley, 2018; Michaud et al., 2018, references therein). Entomopathogens such as Beauveria bassiana (Balsamo) Vuillemin (Ascomycota: Hypocreales) and Lecanicillium spp. (formerly Verticillium lecanii) (Hypocreales: Cordycipitaceae), have also been shown or implicated in having antagonistic activity against both insect pests (Lewis and Cossentine, 1986; Cherry et al., 2004) and plant pathogens (Clark et al., 2006). As such, these fungi present opportunities for multiple use of in integrated pest management strategies (Summers, 1998).
Interactions Between The Plant and Its Biotic Partners: M. Sativa As a Holobiont
Plant-Insect Interactions
The relationship between plants and their insect herbivores has a long evolutionary history (Labandeira, 2013), and one that has been described as an evolutionary “arms race” between plants and herbivorous insects. In response to insect attack, plants have developed physical and chemical defenses to prevent or mitigate feeding and/or oviposition (Trumble et al., 1993; Schardl et al., 2013; Meiners, 2015; Zhang and Li, 2019). Physical defenses include slippery or sticky plants surfaces, trichomes, waxy cuticles, hardness, and architecture to deter feeding (Small, 1996; Whitney and Federle, 2013). Plant chemical protection includes plant secondary defenses that are inducible, and not only protect the plant from UV-light, desiccation or cold, but also from insect herbivores, fungi, bacteria, and viruses (Hartmann, 1996). Medicago sativa possess both biochemical (Agrell et al., 2003; Julier et al., 2004; Wu et al., 2021) and physical defenses (Lovinger et al., 2000) against insect pests. However, the relationship is not often explored beyond the plant-pest level and ignores the relationship to abiotic (soil fertility, moisture, heat stress) and biotic (soil microbiome, endophytes) variables. Tritrophic interactions between plants, insect herbivores, and their natural enemies provides another level of complexity to plant defenses and act to mitigate the impacts of insect herbivores, or biocontrol agents. Herbivore-induced plant volatiles (HIPVs) play a key role in these interactions, as they can attract insect predators and parasitoids to herbivore-attacked plants (Turlings and Erb, 2018). This signaling occurs both above and below ground, and herbivore-damaged roots shown to produce volatiles that attract entomopathogenic nematodes (Rasmann et al., 2005).
More recently, it has been shown that the soil microbiome may also play an important role in plant defenses against above- and below-ground insect herbivory (Howard et al., 2020). Pineda et al. (2019) demonstrated that the composition of the soil microbiome reduced pupation of thrips, Frankliniella occidentalis (Pergande), placed on chrysanthemum leaf cuttings. Conversely, above ground herbivory has been shown to alter the soil microbiome, but the effects were conditional on plant type, soil-microbial community, soil fertility and temporal changes (French et al., 2021; Sveen et al., 2021).
Insect Response to Secondary Metabolites
In response to plant secondary compounds, insects have evolved strategies to overcome these defenses including the ability to suppress or avoid plant stress responses, including suppression of herbivore induced plant volatiles (HIPVs) (Turlings and Erb, 2018). Endosymbionts, specialized bacteria found in insects–are important in allowing the insect to utilize otherwise unsuitable host plants by enabling digestion of plant polymers, detoxification of plant-produced toxins, provisioning of essential nutrients, and providing protection from parasitoids and pathogens (Frago et al., 2012; Hansen and Moran, 2014; Oliver and Martinez, 2014). Endosymbionts have also been shown to protect their insect hosts from abiotic stress (Guo et al., 2017; Lemoine et al., 2020).
Trichoderma
Trichoderma (Ascomycetes, Hypocreales) spp. are cosmopolitan free-living fungi found in foliar environments, soil, fungal material, decaying wood and sediment (Kubicek et al., 2003; Harman et al., 2004; Jaklitsch and Voglmayr, 2015). Their value to the host plant is protection from fungal pathogens, increased plant nutrient uptake, solubilization of soil nutrients, and induction of systemic resistance (Harman et al., 2004; Guzmán-Guzmán et al., 2019). Secretion of effector proteins and secondary metabolites (β-glucanases, harzianolide) by Trichoderma are understood to mediate the beneficial interaction between Trichoderma and plants (Guzmán-Guzmán et al., 2019). Defensive interactions have also been indicated at both the biochemical and molecular level, the defense response potentially fluctuating between induced systemic resistance (ISR) and systemic acquired resistance (SAR), depending on the Trichoderma strain and plant species, as well as biotic and abiotic conditions (Nawrocka and Ma.olepsza, 2013). A small pot experiment looking at the effect of Trichoderma harzianum Rifai on M. sativa growth with and without cutting, found significant increases in plant shoot and root dry weights of mowed plants treated with T. harzianum, compared with the control unmown plants treated with T. harzianum (Zhang et al., 2019). Soil available nutrients (N, P, K) were also elevated compared to the control. More recent research has highlighted the presence of mycovirus in Trichoderma (Lee et al., 2017; Wu et al., 2020). Mycoviruses have been shown to exhibit greater genetic diversity and host range than previously thought, with the ability to move easily between fungal hosts belonging to unrelated (distant) taxonomic groups (Nerva et al., 2017). Although the role of mycovirus in Trichoderma remains unclear, it has been shown that infected Trichoderma can mediate antifungal activity against plant pathogens (Chun et al., 2020).
Plant-Pathogen Interactions
Plants have also been shown to exhibit defensive priming, whereby stimuli from pathogens, beneficial microbes or arthropods, can lead to enhanced activation of induced defense mechanisms throughout the plant. When the plant is subsequently challenged, there is a faster and/or stronger reaction to attack (Maleck and Dietrich, 1999; Mauch-Mani et al., 2017). This adaptive strategy improves the defensive capacity of plants and can involve physiological changes, increased transcriptome and metabolic levels, and epigenetically modulated changes in gene expression. Upon subsequent challenge, the plant effectively mounts a faster and/or stronger defense response that results in increased resistance and/or stress tolerance (Mauch-Mani et al., 2017). Perhaps not surprisingly, plant defense responses can be complex, with both antagonistic and co-ordinated interactions shown to occur between defensive pathways (Maleck and Dietrich, 1999), as well as trade-offs between growth and defense functions (Bastias et al., 2021).
Plant-Endophyte Interactions
The term ‘endophyte’ describes (micro)organisms living inside plant tissues including roots, leaves, stems, flowers, and seeds (Pinski et al., 2019), and can be both bacteria and fungi. In a strict sense, an endophyte must not be detrimental to its host plant (see Lodewyckx et al., 2002 for definitions), but the plant receives an ecological benefit from the presence of the symbiont (Quispel, 1992). In most cases, these benefits include better germination (Ulloa-Muñoz et al., 2020), mobilization of nutrients (Khalifa et al., 2016), and antagonistic effects toward phytopathogens (Pinski et al., 2019; Whitaker and Bakker, 2019).
While research on endophyte bioactivity has focussed on fungi (Arnold et al., 2003; Strobel, 2018), bacterial endophytes, especially those with entomopathogenic activity, are an emerging research area. This is against a backdrop of an increasing failure of conventional agricultural practices (Wemheuer et al., 2016; Le Cocq et al., 2017) and pest management systems (Lewis et al., 1997; Summers, 1998) to provide sustainable food production systems.
A more novel utilization of endophytes is in bioprospecting to identify biologically active compounds with antibiotic, antioxidant, antiparasitic, or cytotoxic activities, or the facilitation of reactive dye discoloration, where endophytes are used as the main produces rather than the plant itself (Savi et al., 2019). For a more in-depth overview on the concept of endophytism and the various beneficial interactions between endophytes and their host-plants and their impact on agriculture and, ultimately, human health, refer to the comprehensive review by Khare et al. (2018).
Both bacterial and fungal endophytes have been isolated from M. sativa (Hardoim et al., 2008; López et al., 2018; Chen et al., 2020), but there is a paucity of research that has examined the stability and prevalence of these endophytes in the plant. For example, one of problems for M. sativa cultivation is growth under drought and salinity stress (Noori et al., 2018). Bacterial endophytes related to Klebsiella, Kosakonia, and Sinorhizobium found in M. sativa root nodules have demonstrated a positive effect on resistance to salinity stress, and provide the potential to extend the arable climate zones for M. sativa. Halomonas and Bacillus endophytes isolated from plants that are capable of growth in high salinity, have been shown to colonize M. sativa and enable seedling growth in the presence of up to 1% NaCl, a non-permissible salt concentration for non-inoculated plants (Kearl et al., 2019).
Plant-Endophyte-Large Herbivore Interactions
Induced responses in plants, initiated by herbivory, create potential for trait-mediated indirect interactions among herbivores, with saliva shown to alter plant response to feeding, which may benefit plant pathogens or insect herbivores. Research by Liu et al. (2016) on the transcriptome response of two-week-old M. sativa seedlings following application of cow saliva to cut leaves, indicated negative effects on regrowth, as well as modification of jasmonic acid synthesis, potentially enhancing the susceptibility to pathogens. Artificial cutting of perennial ryegrass (Lolium perenne L.) leaves induced synthesis of an Epichloë endophyte alkaloid, which reduced feeding by adult Argentine stem weevil [Listronotus bonariensis (Kuschel)]. However, this effect was mitigated by sheep (Ovis aries L.) saliva applied to the cut sections (Bultman et al., 2018). The authors speculated that the observed effect may involve salivary enzymes or perhaps even metabolites from living microbes within the saliva. Moose (Alces alces L.) browsing on Scots pine (Pinus sylvestris L.) were found to improve the performance of the sawfly (Neodiprion sertifer Geoffroy) compared to unbrowsed trees (Nordkvist et al., 2019). Interestingly, the compound di-terpene, known to affect sawfly performance, was not affected by the browsing treatments nor correlated with sawfly performance parameters, suggesting other secondary compounds were active in the response.
Plant secondary compounds can also have direct impacts on large herbivores. Coumestrol, a phytoestrogen compound is naturally found in M. sativa, and if elevated in the leaves, can have detrimental impacts on sheep reproductivity (Fields et al., 2018). Foliar fungal infection or aphid feeding have both been shown to increase the concentration of coumestrol, sometimes to levels that could interfere with ewe reproductive performance (Kain and Biggs, 1980; Purves et al., 1981; Fields et al., 2018). Toxins associated with endophyte (Epichloë species; Clavicipitaceae) commonly associated with cool season grasses (Poaceae, subfamily Poöideae), not only have activity against insects but also vertebrates (Johnson et al., 2013; Schardl et al., 2013; Hume et al., 2016). However, whether bacterial endophytes associated with M. sativa have an impact on large herbivores is unknown.
Multitrophic Interactions
Tri-trophic relationships have also been shown to be intricate in terms of plant signaling and concomitant response from natural enemies. A study investigating plant host effect and parasitism by the endoparasitoid Diaeretiella rapae (M'Intosh) on cabbage aphid, Brevicoryne brassicae (L.) genotypes, found that plant cultivar had significant effect on aphid reproduction, rates of parasitism and parasitoid sex ratio (Mehrparvar et al., 2019). As B. brassicae lacks facultative endosymbionts that may provide protection from parasitism (Clark et al., 2012), the effect on D. rapae reported by Mehrparvar et al. (2019) cannot be attributed to known defensive endosymbionts. However, as found with A. pisum, the effect of host plant on the incidence of the defensive endosymbiont Hamiltonella defensa, and associated parasitism rates can be minimal (Sochard et al., 2019; Smith et al., 2021). Insect herbivore-induced plant defenses are well-known to influence the abilities of baculoviruses to infect, replicate in, and kill their insect hosts (Ali et al., 1998; Shikano et al., 2017). Helicoverpa zea (Boddie) (Lepidoptera: Noctuidiae) caterpillars treated with a sublethal dose of baculovirus and feeding on tomato (Solanum lycopersicum L.) plants, elicited the highest plant anti-herbivore defense compared to plant responses to healthy caterpillars (Pan et al., 2019). However, it was not known if the increase in plant defenses induced by virus-infected caterpillars benefited the virus or the caterpillar (Pan et al., 2019). The use of volatiles that attract natural insect pest enemies has been suggested as a way of controlling important agricultural pests through a push-pull strategy (Pickett and Khan, 2016) and has been successfully used in maize against lepidopteron stem borers (Pickett et al., 2014). Increases in plant peroxidase (POD) and catalase (CAT) activities, along with salicylic acid (SA) concentration, have been suggested as an explanation for enhanced defense response to A. pisum in M. sativa inoculated with the mycorrhizal fungi Rhizophagus intraradices (N.C.Schenck & G.S.Sm.) C.Walker & A.Schüssler (Li et al., 2019).
Factors Impacting These Interactions
Climate Change
While the environmental impacts of climate change are complex (Pedrono et al., 2016; Yang et al., 2021), current and future predictions indicate that crop losses associated with insect pests, plant pathogens, and weeds, will become more frequent and substantial (Baker et al., 2015; Deutsch et al., 2018; Anderson et al., 2020). This includes shifts in insect distribution (Battisti and Larsson, 2015; Ricciardi et al., 2021), loss of biodiversity (Bellard et al., 2014), and associated impacts on ecosystem services (Pedrono et al., 2016). There will also be increased biosecurity impacts as pathways and vectors associated with trade and tourism provide the means to move high impact pests and diseases, rapidly and across vast distances (McNeill et al., 2021; Ricciardi et al., 2021).
The effects of climate change, particularly elevated CO2 and temperature, and water availability on M. sativa production and persistence, has been and continues to be an area of significant research. Research has demonstrated both positive and negative impacts on photosynthesis, respiration, nodulation function and efficiency, biomass allocation, growth and quality of M. sativa, which are covered in the reviews by Liang et al. (2013), Aranjuelo et al. (2014), and Soares et al. (2019).
Elevated CO2 levels will also change insect phenology and their impact parameters. High carbon:nitrogen (C:N) ratios associated with increased CO2 levels have been shown to affect the performance of insect herbivores positively (aphids) but to be mostly neutral for chewing insects (Whittaker, 1999). Aphids grown at high CO2 levels were found to be more responsive to aphid alarm pheromones, potentially making them more vulnerable to parasitism (Awmack et al., 1997). Elevated CO2 was also shown to increase the duration of nymphal stages, decrease adult longevity and fecundity of A. pisum (Li C. et al., 2021). The researchers also found changes in the nutritional profiles of the aphid, with a significant interaction between CO2 levels, and nymph generations.
A study on above- and below-ground herbivory on white clover (T. repens L.) by clover root weevil (Sitona obsoletus, (Gmelin), formerly S. lepidus) found that elevated CO2 resulted in significantly increased root C:N ratios, increases in root mass (85% greater) and nodule abundance (220% more abundant) (Johnson and McNicol, 2010). Adult S. obsoletus consumed significantly more foliage but laid fewer eggs under elevated CO2. Conversely, there was increased larval abundance and performance at elevated CO2, which was positively correlated with the number of nodules available (Johnson and McNicol, 2010). The authors concluded that reduced foliar quality at elevated CO2 was generally disadvantageous for adult S. obsoletus living above-ground, but very beneficial for larvae feeding on the root system, due to enhanced nodulation (Johnson and McNicol, 2010). Research to determine if this response is found with other Sitona species known to feed on M. sativa (e.g., S. discoideus Gyllenhal, S. hispidulus F.) has yet to be undertaken.
Climate change may impact the seasonal ecology of parasitoids, with consequences on host–parasitoid synchrony and population cycles, food-web functioning, and ecosystem services such as biological pest control (Jamieson et al., 2012; Tougeron et al., 2020). Research on the effect of abiotic and biotic factors on larch casebearer [Coleophora laricella, (Hübner)] defoliation of larch (Larix spp.) and its two parasitoids Agathis pumila (Ratzeburg) and Chrysocharis laricinellae (Ratzeburg), found that warming temperatures disrupted the complex interaction between trophic levels possibly contributing to casebearer outbreaks in North America (Ward et al., 2020). The broad concepts discussed above for both above- and below- ground interactions are shown in Figure 1.
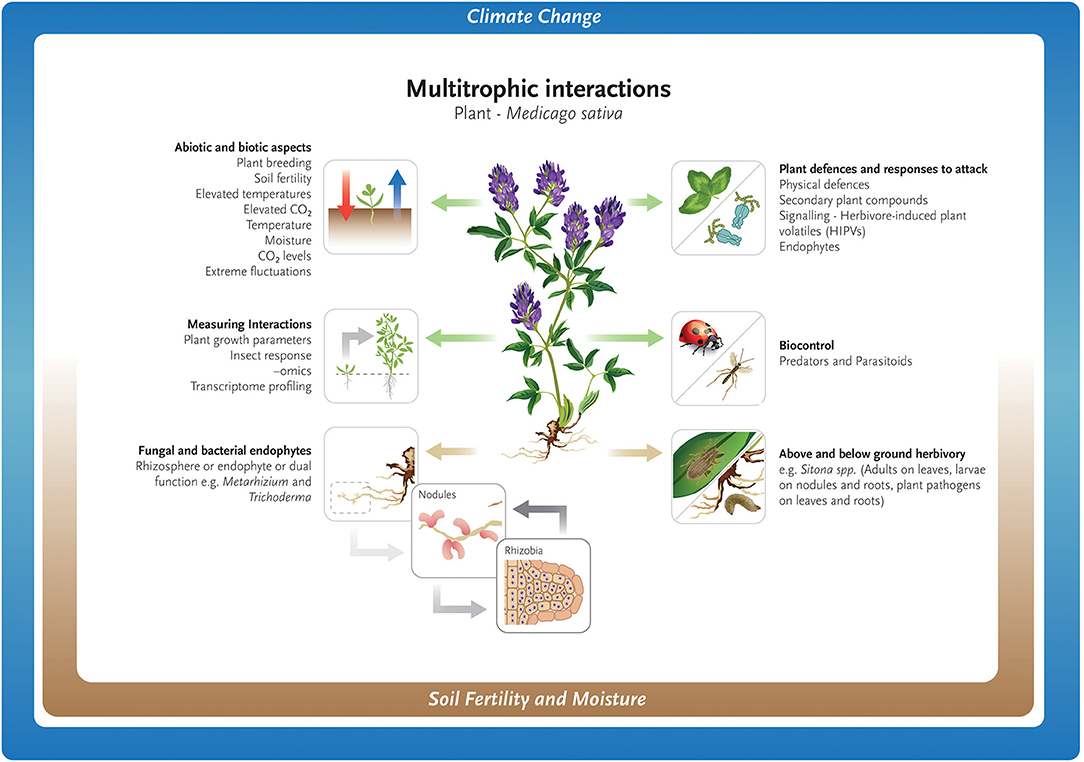
Figure 1. Abiotic and biotic challenges for the growth and maintenance of Medicago sativa as a forage crop.
Genotype
Despite the evident importance of endophytes–bacterial and fungal–little is known about the genetic background that drives the evolution of the plant-endophyte-pathogen relationship. Plasmids are a recognized key driver of rapid evolution, providing the means to acquire genes encoding novel metabolic pathways, or gain resistance toward metals and antibiotics via horizontal gene transfer (Schierstaedt et al., 2019). Similarly, genes related to pathogenicity and host defense can be acquired through plasmid encoded genes. However, to fully understand the complex network of communication between host plants, endophytes and pathogens/predators, it is necessary to take on a holistic approach that encompasses many different-Omic disciplines rather than pursuing a reductionist view and investigating individual components in isolation (Kaul et al., 2016; Pinski et al., 2019). Genome sequencing and subsequent comparative genome analyses will provide the necessary foundation to develop novel hypotheses to be validated by a wide spectrum of -omics technologies. While research on the genetic blueprint is slowly emerging, it often is limited to the investigation of microbiome phylogeny via 16S rRNA and housekeeping gene sequencing (Kumar et al., 2018; Liotti et al., 2018; Kang et al., 2019) or high-level biochemical characterization via proteome and metabolome studies (López et al., 2018). Genomic analysis of Bacillus spp. strains has been proposed as being critical to understanding the complex and intricate plant host-microbiome-(insect) pathogen communication network (Lopes et al., 2018). Transcriptomics datasets enable the study of changes in gene expression over time, as endophytes and their hosts encounter environmental changes such as insect pests. However, the varying levels of gene expression do not reveal the function of the resulting proteins and enzymes and proteomic/metabolomic approaches are required to relate the genomic diversity to the functionality of microbes (Rasmussen et al., 2012; Afroz et al., 2013). Studies into the M. sativa interactome first emerged a decade ago (Rodriguez-Llorente et al., 2009). These described the symbiosis interactome between the model bacterium Sinorhizobium meliloti with its legume host using computational methods and models that provided a theoretical basis, but lacked experimental validation.
Production and Persistence
As a forage crop with a worldwide distribution, breeding of germplasm to adapt to a range of climates has been essential to achieve sustainable high quality forage production for livestock (Undersander et al., 2011). Conventional plant breeding techniques (e.g., selective breeding, cross breeding, male sterile line breeding), have targeted yield, quality, persistence under repeated grazing or cutting, heat and cold tolerance, salinity and salt tolerance, drought, high moisture, and insect pest and disease resistance (Bouton, 2012; Scasta et al., 2012; Annicchiarico et al., 2015; Shi et al., 2017). Selection of salinity-tolerant actinobacteria has been demonstrated to improve rhizobia function and overall M. sativa production under salt stress (Saidi et al., 2021). As the demand for meat-based proteins increases in response to human population growth, so too has the environmental limits under which M. sativa is being grown. This has led to evaluation of genotypes adapted for subtropical conditions (Acharya et al., 2020; Hoppen et al., 2022), for both high altitude and latitude (Shi et al., 2017), and environments with high temperature and low soil moisture (del Pozo et al., 2017; Lemaire et al., 2019).
Environment plays a critical role to determining yield and persistence (Li et al., 2010). Pembleton et al. (2010) showed that under a cool temperate climate, environment had a significant impact on the persistence and yield of alfalfa genotypes, with winter active genotypes having low persistence and DM yield. Breeding for grazing tolerance has been shown to be important in persistence (Sewell et al., 2011; Harvey et al., 2014; Burnett et al., 2020), but there can be a trade off in production, with winter active germplasm generally showing poor persistence (Humphries et al., 2006; Harvey et al., 2014). What is apparent, is that as the worldwide range of M. sativa expands, breeding programs to meet regional conditions will become more important (Bouton, 2012; Shi et al., 2017).
Future Research
This review has examined several aspects of plant-insect-microbe-interaction, and highlighted their complexity. In relation to M. sativa, there are research opportunities to address important gaps in our understanding of the biotic and abiotic parameters that can be exploited to improve the significance and utility of the plant. This includes the role of endophytes on growth, colonization and transmission, subsequent persistence, their prevalence in M. sativa and their impact on insect herbivory and pathogens. Bioprospecting for endophyte strains that mediate resistance outside cultivated strains would also seem worthwhile. Medicago sativa is believed to have originated in the Caucasus region: north-eastern Turkey, Turkmenistan and north-western Iran (Michaud et al., 1988). Surveys in these regions may yield valuable “wild-type” germplasm containing bacterial endophytes or traits that provides tolerance or resistance to certain pests and diseases. China also has potential unique M. sativa germplasm (Shi et al., 2017), that may provide ecotype–endophyte associations with protection from insect pests and diseases. However, while this approach would intuitively seem a useful strategy, it may not necessarily yield the desired outcome. For instance, M. sativa and alfalfa weevil (H. postica) are sympatric in Turkey, but a search for plants naturally resistant to the weevil were unsuccessful (Ratcliffe and Campbell, 1995), although resistant germplasm has been reported in Iran (Abbasi, 2020). Other factors also need consideration, including the abiotic environment and its influence on plant responses to pests and pathogens. Increasing agricultural intensification has been found to have a strong negative association with root fungal communities (Banerjee et al., 2019). Fertilizer application may have a negative effect on bacterial endophytes associated with M. sativa, as has been demonstrated in grasses (Wemheuer et al., 2016). Conversely, rhizobia need critical mineral nutrients for metabolic processes to enable their survival and growth as free-living soil saprophytes, and in their symbiotic relationship with legumes (O'Hara, 2001). Non-rhizobia found in the rhizosphere and nodule microbiome are also critical to nodule formation and legume fitness (Schaedel et al., 2021). The impact of grazing or cutting on the ability of M. sativa to respond to pest and pathogen attack are also areas for potential research.
Climate change presents an obvious challenge, not only because there will be more instability in climatic events such as drought or floods, but economically valuable plant species will potentially be exposed to new pests and plant diseases due to range expansion, (Trebicki et al., 2017; Ricciardi et al., 2021), either through natural dispersal or spread along trade and tourism pathways (McNeill et al., 2021). Elevated temperatures will have both negative and positive consequences for biological control (Thomson et al., 2010; Gerard et al., 2013), and plant defense responses to pathogens (Venkatesh and Kang, 2019). Creating crop varieties that are highly attractive to natural pest enemies has been suggested as a simple way to achieve better biological control of pests (Dhandaydham et al., 2008; Dicke and Baldwin, 2010). Beyond traditional plant breeding approaches (Annicchiarico et al., 2015; Capstaff and Miller, 2018), gene editing technology has been proposed as a way of improving the adaptability of plants to challenging abiotic and biotic conditions (Sanzari et al., 2019; Demirer et al., 2021). Transgenic plants with desirable traits for growth or pest resistance are already available (e.g., cotton or maize) and applicable to M. sativa. For example, under laboratory conditions Wang et al. (2019) demonstrated that by insertion of a gene expressing an aphid alarm pheromone into M. sativa, repellency by A. pisum to transformed plants was shown in subsequent behavioral tests. Technologies such as CRISPR/Cas genome editing (Cobb et al., 2019; Pixley et al., 2019), nanotechnologies (Sanzari et al., 2019; Behl et al., 2022), or the combination of both nanotechnologies with CRISPR-Cas technology (Demirer et al., 2021), has also been suggested as a means of improving plant production, quality, and persistence under challenging environments. Nanotechnology may also provide a means of monitoring crops by translating plant chemical signals into digital information that can be monitored by standoff electronic devices (Giraldo et al., 2019). While mainly conceptual, synthetic engineering of the phytomicrobiome has also been suggested as a way of improving plant performance (Ke et al., 2021). These new technologies will also require both regulatory and social license to operate, as well as biological-based research to understand relationships and benefits across trophic levels and different environments (Sanzari et al., 2019; Behl et al., 2022). Understandably, adoption of these technologies will be variable on a global scale as dictated by government and public timeframes.
Although some progress has been made to increase insect resistance to crops, research on M. sativa still provides opportunities for improvement in this important field. Most notably, the impact of bacterial endophytes on plant resistance to pests and pathogens is yet to be fully explored, despite the attractiveness of this target with regards to rapid evolution and genetic engineering (Figure 2). This knowledge gap opens significant opportunities in the future for new research that focuses on unraveling the genetic and metabolic networks between bacterial endophytes, host plants and insect pest and beneficial species, with the aim to engineer or naturally evolve bacterial endophytes with enhanced insecticidal properties. As a first step and to build the fundamentally required genomic knowledge base, a comprehensive catalog of genomes of known M. sativa bacterial endophytes needs to be established, preferably from microbiomes isolated from a range of agricultural M. sativa growth regions worldwide and wild-type relatives. Based on this foundation, transcriptomic and metatranscriptomic analyses will unravel how bacterial gene expression changes over time and to plant and insect exposure. In a second step, changes in gene expression levels of the host plant and insect predators can be quantified and correlated to bacterial endophytes. Novel insights gained from these experiments will be critical in understanding key genetic building blocks of insect resistance. Metaproteomic and metabolomics studies will translate the genetic basis into identifying the presence, absence and modification of biologically active compounds, and their concomitant effects on both plant and associated insect pests (Zogli et al., 2020). Once the intricate network of interactions and metabolic responses is better understood, bacterial endophyte strains may be directly evolved toward specific metabolic changes that will specifically enhance insect resistance–or target other limitations of M. sativa cultivation such as drought or salinity.
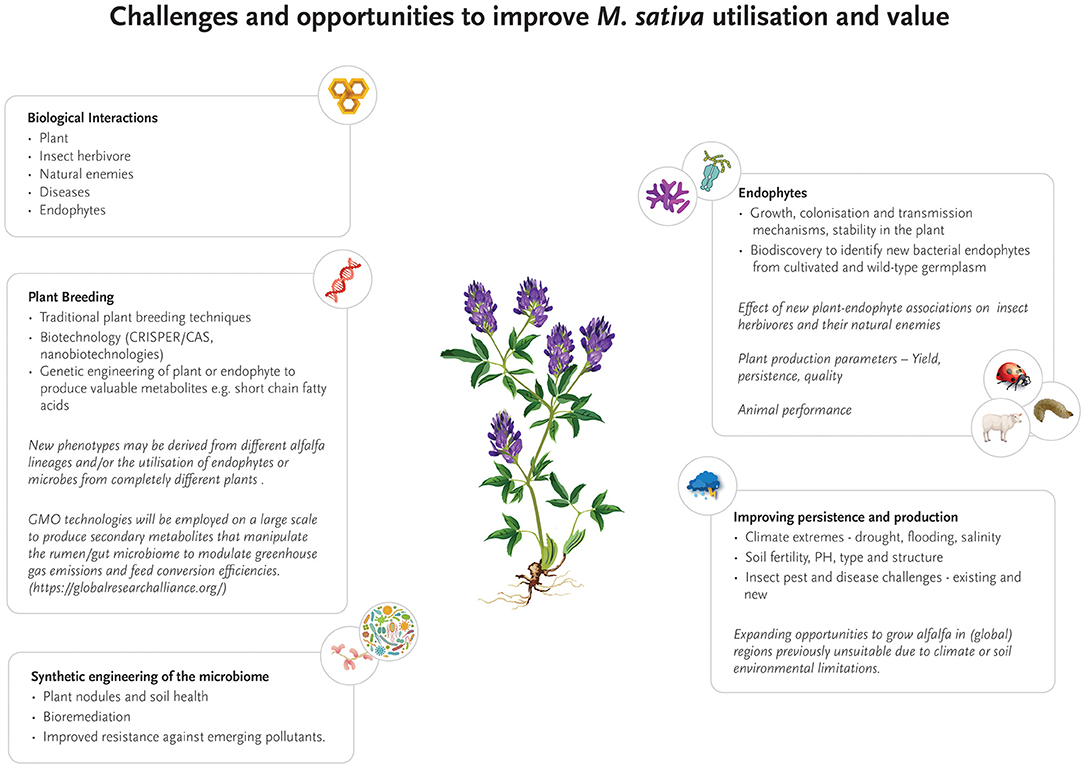
Figure 2. Current and future research opportunities to improve the production, persistence, quality and geographic range of Medicago sativa as a forage crop.
Conclusion
A broader, inter-disciplinary approach that simultaneously addresses plant-insect-endophyte-plant pathogen interaction and their impacts viewed against abiotic and biotic aspects is desirable, as it concurrently addresses a range of research questions (Raffa et al., 2020). The benefits are research advances and outcomes that support agricultural resilience, industry stakeholders and farmers. However, achieving this aim obviously depends on appropriate research to allow M. sativa to achieve its potential in a challenging environment.
Author Contributions
MM, XT, EA, WB, and SS contributed to the development of the concepts discussed in this review. All authors contributed to writing the manuscript. All authors contributed to the article and approved the submitted version.
Funding
The contribution of MM and EA was funded by AgResearch via the AgResearch Foresight Developing New Science Connections programme (PRJ0271178). The contribution of XT was funded by China Agriculture Research System of MOF and MARA(CARS-34). The contribution of SS was supported via AgResearch Strategic Science Investment Fund via the New Zealand Ministry of Business Innovation and Employment (Contract No. C10X1702 and A25344 Microbiomes from soils to plate).
Conflict of Interest
The authors declare that the research was conducted in the absence of any commercial or financial relationships that could be construed as a potential conflict of interest.
Publisher's Note
All claims expressed in this article are solely those of the authors and do not necessarily represent those of their affiliated organizations, or those of the publisher, the editors and the reviewers. Any product that may be evaluated in this article, or claim that may be made by its manufacturer, is not guaranteed or endorsed by the publisher.
Acknowledgments
The authors would like to thank Karen Cousins (AgResearch Ltd.) for assistance in the literature search and Alice Baillie (AgResearch Ltd.) for the figure graphics. We also thank AgResearch internal referees Joanne Jensen and Richard Johnson and referees for helpful suggestions and comments during journal review. We would like to acknowledge support from the AgResearch Strategic Science Investment Fund via the New Zealand Ministry of Business Innovation and Employment (Contract No. C10X1702 and A25344 Microbiomes from soils to plate) and the Better Border Biosecurity (B3) project (Contract No. A25757 Biosecurity over the horizon).
References
Abbasi, M. R. (2020). Resistance to the alfalfa weevil in the Iranian collection of Medicago sativa. Trop. Grassl. 8, 263–279 doi: 10.17138/tgft(8)263-279
Acharya, J. P., Lopez, Y., Gouveia, B. T., de Bem Oliveira, I., Resende, M. F. R. Jr., Muñoz, P. R., and Rios, E. F. (2020). Breeding alfalfa (Medicago sativa L.) adapted to subtropical agroecosystems. Agronomy. 10, 742. doi: 10.3390/agronomy10050742
Afroz, A., Zahur, M., Zeeshan, N., and Komatsu, S. (2013). Plant-bacterium interactions analyzed by proteomics. Front. Plant Sci. 4, 21-21. doi: 10.3389/fpls.2013.00021
Agrell, J., Oleszek, W., Stochmal, A., Olsen, M., and Anderson, P. (2003). Herbivore-induced responses in alfalfa (Medicago sativa). J. Chem Ecol. 29, 303–320 doi: 10.1023/A:1022625810395
Ali, M. I., Felton, G. W., Meade, T., and Young, S. Y. (1998). Influence of interspecific and intraspecific host plant variation on the susceptibility of heliothines to a baculovirus. Biol. Control. 12, 42–49. doi: 10.1006/bcon.1998.0619
Anderson, R., Bayer, P. E., and Edwards, D. (2020). Climate change and the need for agricultural adaptation. Curr. Opin. Plant Biol. 56, 197–202. doi: 10.1016/j.pbi.2019.12.006
Annicchiarico, P. (2007). Lucerne shoot and root traits associated with adaptation to favourable or drought-stress environments and to contrasting soil types. Field Crops Res. 102, 51–59. doi: 10.1016/j.fcr.2007.01.005
Annicchiarico, P., Barrett, B., Brummer, E. C., Julier, B., and Marshall, A. H. (2015). Achievements and challenges in improving temperate perennial forage legumes. Crit. Rev. Plant Sci. 34, 1–3, 327–380, doi: 10.1080/07352689.2014.898462
Aranjuelo, I., Arrese-Igor, C., and Molero, G. (2014). Nodule performance within a changing environmental context. J. Plant Physiol. 171, 1076–1090. doi: 10.1016/j.jplph.2014.04.002
Arnold, A. E., Mejía, L. C., Kyllo, D., Rojas, E. I., Maynard, Z., Robbins, N., et al. (2003). Fungal endophytes limit pathogen damage in a tropical tree. Proc. Natl. Acad. Sci. U.S.A. 100, 15649–15654. doi: 10.1073/pnas.2533483100
Avery, D., Avery, F., Ogle, G. I., Wills, B. J., and Moot, D. J. (2008). Adapting farm systems to a drier future. Proc. New Zealand Grassl. Assoc. 70, 13–18. doi: 10.33584/jnzg.2008.70.2710
Awmack, C. S., Woodcock, C. M., and Harrington, R. (1997). Climate change may increase vulnerability of aphids to natural enemies. Ecol. Entomol. 22, 366–368. doi: 10.1046/j.1365-2311.1997.00069.x
Baker, M. B., Venugopal, P. D., and Lamp, W. O. (2015). Climate change and phenology: Empoasca fabae (Hemiptera: Cicadellidae) migration and severity of impact. PLoS ONE 10, e0124915. doi: 10.1371/journal.pone.0124915
Banerjee, S., Walder, F., Büchi, L., Meyer, M., Held, A. Y., Gattinger, A., et al. (2019). Agricultural intensification reduces microbial network complexity and the abundance of keystone taxa in roots. ISME J. 13, 1722–1736. doi: 10.1038/s41396-019-0383-2
Bastias, D. A., Gianoli, E., and Gundel, P. E. (2021). Fungal endophytes can eliminate the plant growth-defence trade-off. New Phytol. 230, 2105–2113. doi: 10.1111/nph.17335
Battisti, A., and Larsson, S. (2015). “eClimate change and insect pest distribution range f,” in Climate Change and Insect Pests, eds. C. Bjorkman, and P. Niemela (Wallingford: CABI Int), 1–15.
Behl, T., Kaur, I., Sehgal, A., Singh, S., Sharma, N., Bhatia, S., et al. (2022). The dichotomy of nanotechnology as the cutting edge of agriculture: nano-farming as an asset versus nanotoxicity. Chemosphere. 288, 132533. doi: 10.1016/j.chemosphere.2021.132533
Bellard, C., Leclerc, C., Leroy, B., Bakkenes, M, Veloz, S, Thuiller, W., et al. (2014). Impacts of climate change on the future of biodiversity. Global Ecol. Biogeogr. 23, 1376–1386. doi: 10.1111/geb.12228
Bouton, J. H. (2012). An overview of the role of lucerne (Medicago sativa L.) in pastoral agriculture. Crop Pasture Sci. 63, 734–738. doi: 10.1071/CP12127
Bultman, T. L., McNeill, M. R., Krueger, K., De Nicolo, G., Popay, A. J., Hume, D. E., et al. (2018). Complex interactions among sheep, insects, grass, and fungi in a simple New Zealand grazing system. J. Chem. Ecol. 44, 957–964. doi: 10.1007/s10886-018-0993-6
Burnett, V. F., Butler, K. L., Hirth, J. R., Mitchell, M. L., Clark, S. G., and Nie, Z. (2020). Lucerne (Medicago sativa L.) persistence remains unchanged under variable cutting regimes. Agronomy. 10, 844. doi: 10.3390/agronomy10060844
Cameron, P. J., Sunde, R. G., and Walker, G. P. (1983). Discovery, identification, and host plant interaction of spotted alfalfa aphid (Hemiptera: Aphididae) in New Zealand. New Zealand J. Agric. Res. 26, 511–517. doi: 10.1080/00288233.1983.10427030
Cameron, P. J., and Walker, G. P. (1989). Release and establishment of Aphidius spp. (Hymenoptera: Aphidiidae), parasitoids of pea aphid and blue green aphid in New Zealand. New Zealand J. Agric. Res. 32, 281–290. doi: 10.1080/00288233.1989.10423463
Capstaff, N. M., and Miller, A. J. (2018). Improving the yield and nutritional quality of forage crops. Front. in Plant Sci. 9, 535. doi: 10.3389/fpls.2018.00535
Carlsson, G., and Huss-Danell, K. (2003). Nitrogen fixation in perennial forage legumes in the field. Plant and Soil. 253, 353–372. doi: 10.1023/A:1024847017371
Chen, X.-L., Li, M., Liu, C.-C., Ma, J., Wang, X., Wu, B.-L., et al. (2020). Exploration of endophytes from alfalfa (Medicago sativa L.) as biocontrol agents. Genet. Genom. 1, 3–8. doi: 10.31487/j.GG.2020.01.01
Cherry, A. J., Banito, A., Djegui, D., and Lomer, C. (2004). Suppression of the stem-borer Sesamia calamistis (Lepidoptera; Noctuidae) in maize following seed dressing, topical application and stem injection with African isolates of Beauveria bassiana. Int. J. Pest Manag. 50, 67–73. doi: 10.1080/09670870310001637426
Chun, J., Na, B., and Kim, D.-H. (2020). Characterization of a novel dsRNA mycovirus of Trichoderma atroviride NFCF377 reveals a member of “Fusagraviridae” with changes in antifungal activity of the host fungus. J. of Microbiol. 58, 1046–1053. doi: 10.1007/s12275-020-0380-1
Clark, E. L., Daniell, T. J., Wishart, J., Hubbard, S. F., and Karley, A. J. (2012). How conserved are the bacterial communities associated with aphids? A detailed assessment of the Brevicoryne brassicae (Hemiptera: Aphididae) Using 16S rDna. Environ. Entomol. 41, 1386–1397. doi: 10.1603/EN12152
Clark, M. M., Gwinn, K. D., and Ownley, B. H. (2006). Biological control of Pythium myriotylum. Phytopath. 96, S25.
Cobb, J. N., Juma, R. U., Biswas, P. S., Arbelaez, J. D., Rutkoski, J., Atlin, G., et al. (2019). Enhancing the rate of genetic gain in public-sector plant breeding programs: lessons from the breeder's equation. Theor. Appl. Genet. 132, 627–645. doi: 10.1007/s00122-019-03317-0
Compendium of Alfalfa Diseases and Pests. (2016). Samac, D.A., Rhodes, L.H., and Lamp, W.O.(eds). 3rd edition. St. Paul, MN: APS Publications.
De Oliveira, E. J., Rabinovitch, L., Monnerat, R. G., Passos, L. K. J., and Zahner, V. (2004). Molecular characterization of Brevibacillus laterosporus and its potential use in biological control. App. Environ. Micro. 70, 6657–6664. doi: 10.1128/AEM.70.11.6657-6664.2004
del Pozo, A., Ovalle, C., Espinoza, S., Barahona, V., Gerding, M., and Humphries, A. (2017). Water relations and use-efficiency, plant survival and productivity of nine alfalfa (Medicago sativa L.) cultivars in dryland Mediterranean conditions. Eur. J. Agron. 84, 16–22. doi: 10.1016/j.eja.2016.12.002
Demirer, G. S., Silva, T. N., Jackson, C. T., Thomas, J. B., Ehrhardt, D. W., Rhee, S. Y., et al. (2021). Nanotechnology to advance CRISPR–Cas genetic engineering of plants. Nat. Nanotechnol. 16, 243–250. doi: 10.1038/s41565-021-00854-y
Deutsch, C. A., Tewksbury, J. J., Tigchelaar, M., Battisti, D. S., Merrill, S. C., Huey, R. B., et al. (2018). Increase in crop losses to insect pests in a warming climate. Science. 361, 916–919. doi: 10.1126/science.aat3466
Dhandaydham, M., Charles, L., Zhu, H., Starr, J. L., Huguet, T., Cook, D. R., et al. (2008). Characterization of root-knot nematode resistance in Medicago truncatula. J. Nematol. 40, 46–54.
Dicke, M., and Baldwin, I. T. (2010). The evolutionary context for herbivore-induced plant volatiles: beyond the 'cry for help'. Trends Plant Sci. 15, 167-175. doi: 10.1016/j.tplants.2009.12.002
Ferguson, C. M., Barratt, B. I. P., Bell, N., Goldson, S. L., Hardwick, S., Jackson, M., et al. (2019). Quantifying the economic cost of invertebrate pests to New Zealand's pastoral industry. New Zealand J. Agric. Res. 62, 255–315. doi: 10.1080/00288233.2018.1478860
Fields, R. L., Barrell, G. K., Gash, A., Zhao, J., and Moot, D. J. (2018). Alfalfa coumestrol content in response to development stage, fungi, aphids, and cultivar. Agron. J. 110, 910–921. doi: 10.2134/agronj2017.09.0535
Frago, E., Dicke, M., and Godfray, H. C. J. (2012). Insect symbionts as hidden players in insect-plant interactions. Trends Ecol. Evol. 27, 705–711. doi: 10.1016/j.tree.2012.08.013
French, E., Kaplan, I., and Enders, L. (2021). Foliar aphid herbivory alters the tomato rhizosphere microbiome, but initial soil community determines the legacy effects. Front. Sustain. Food Syst. 5, 629684. doi: 10.3389/fsufs.2021.629684
Garran, J., and Gibbs, A. (1982). Studies on alfalfa mosaic virus and alfalfa aphids. Australian J. Agric. Res. 33, 657–664. doi: 10.1071/AR9820657
Gerard, P. J., Barringer, J. R. F., Charles, J. G., Fowler, S. V., Kean, J. M., Phillips, C. B., et al. (2013). Potential effects of climate change on biological control systems: case studies from New Zealand. BioControl. 58, 149–162. doi: 10.1007/s10526-012-9480-0
Giraldo, J. P., Wu, H., Newkirk, G. M., and Kruss, S. (2019). Nanobiotechnology approaches for engineering smart plant sensors. Nat. Nanotechnol. 14, 541–553. doi: 10.1038/s41565-019-0470-6
Godfrey, L. D., Legg, D. E., and Yeargan, K. V. (1986). Effects of soil-borne organisms on spring alfalfa establishment in an alfalfa rotation system. J. Econ. Entomol. 79, 1055–1063. doi: 10.1093/jee/79.4.1055
Goldson, S. L., Dyson, C. B., Proffitt, J. R., Frampton, E. R., and Logan, J. A. (1985). The effect of Sitona discoideus Gyllenhal (Coleoptera:Curculionidae) on lucerne yields in New Zealand. Bull. Entomol. Res. 75, 429–442. doi: 10.1017/S000748530001453X
Goldson, S. L., Frampton, E. R., and Proffitt, J. R. (1988). Population dynamics and larval establishment of Sitona discoideus (Coleoptera: Curculionidae) in New Zealand lucerne. J. App. Ecol. 25, 177–195. doi: 10.2307/2403617
Goldson, S. L., and Muscroft-Taylor, K. E. (1988). Inter-seasonal variation in Sitona discoideus Gyllenhal (Coleoptera: Curculionidae) larval damage to lucerne in Canterbury and the economics of insecticidal control. New Zealand J. Agric. Res. 31, 339–346. doi: 10.1080/00288233.1988.10423424
Goldson, S. L., Proffitt, J. R., and McNeill, M. R. (1990). Seasonal biology and ecology in New Zealand of Microctonus aethiopoides (Hymenoptera: Braconidae), a parasitoid of Sitona spp. (Coleoptera: Curculionidae), with special emphasis on atypical behaviour. J. Appl. Ecol. 27(2), 703-722. doi: 10.2307/2404313
Guo, J., Hatt, S., He, K., Chen, J., Francis, F., and Wang, Z. (2017). Nine facultative endosymbionts in aphids. A review. J. Asia-Pacific Ento. 20, 794–801. doi: 10.1016/j.aspen.2017.03.025
Guzmán-Guzmán, P., Porras-Troncoso, M. D., Olmedo-Monfil, V., and Herrera-Estrella, A. (2019). Trichoderma species: Versatile plant symbionts. Phytopath. 109, 6–16. doi: 10.1094/PHYTO-07-18-0218-RVW
Hansen, A. K., and Moran, N. A. (2014). The impact of microbial symbionts on host plant utilization by herbivorous insects. Mol. Ecol. 23, 1473–1496. doi: 10.1111/mec.12421
Hardoim, P. R., Van Overbeek, L. S., and Elsas, J. D. (2008). Properties of bacterial endophytes and their proposed role in plant growth. Trends Microbiol. 16, 463–471. doi: 10.1016/j.tim.2008.07.008
Harman, G. E., Howell, C. R., Viterbo, A., Chet, I., and Lorito, M. (2004). Trichoderma species - Opportunistic, avirulent plant symbionts. Nature Reviews Microbiol. 2, 43–56. doi: 10.1038/nrmicro797
Hartmann, T. (1996). Diversity and variability of plant secondary metabolism: A mechanistic view. Entomol. Exp. Appl. 80, 177–188. doi: 10.1111/j.1570-7458.1996.tb00914.x
Harvey, B., Widdup, K., and Barrett, B. (2014). An evaluation of lucerne for persistence under grazing in New Zealand. Proc. New Zealand Grassl. Assoc. 76, 111–116. doi: 10.33584/jnzg.2014.76.2954
He, Z.-C., Zhang, Z.-L., Huang, F., and Jiang, Y.-W. (1997). A colored pictorial handbook of agricultural pests in northern China. Liaoning Sci. and Technology Press, Shenyang, China 473pp.
Hoppen, S. M., Neres, M. A., and Moot, D. (2022). Factors related to productivity and persistence of lucerne (Medicago sativa) genotypes with different fall dormancy levels: a review. Res. Soc. Develop. 11, e11711124473. doi: 10.33448/rsd-v11i1.24473
Howard, M. M., Muñoz, C. A., Kao-Kniffin, J., and Kessler, A. (2020). Soil microbiomes from fallow fields have species-specific effects on crop growth and pest resistance. Front. in Plant Sci. 11, 1171. doi: 10.3389/fpls.2020.01171
Huang, Z., Liu, Y., Cui, Z., Fang, Y., He, H.-H., Liu, B.-R., et al. (2018). Soil water storage deficit of alfalfa (Medicago sativa) grasslands along ages in arid area (China). Field Crops Res. 221, 1–6. doi: 10.1016/j.fcr.2018.02.013
Hume, D. E., Ryan, G. D., Gibert, A., Helander, M., Mirlohi, A., and Sabzalian, M. R. (2016). “Epichloë fungal endophytes for grassland ecosystems”, in Sustainable Agriculture Reviews, Lichtfouse, E., (eds). Cham: Springer International Publishing. p. 233-305. doi: 10.1007/978-3-319-26777-7_6
Humphries, A. W., Kobelt, E. T., Bellotti, W. D., and Auricht, G. C. (2006). Tolerance of Australian lucerne (Medicago sativa) germplasm to grazing by sheep. Aust. J. Exp. Agric. 46, 1263–1270. doi: 10.1071/EA04044
Jaber, L. R., and Ownley, B. H. (2018). Can we use entomopathogenic fungi as endophytes for dual biological control of insect pests and plant pathogens? Biol. Control 116, 36-45. doi: 10.1016/j.biocontrol.2017.01.018
Jackson, T. A., Townsend, R. J., Dunbar, J. E., Ferguson, C. M., Marshall, S. D. G., and Zydenbos, S. M. (2012). Anticipating the unexpected - managing pasture pest outbreaks after large-scale land conversion. Proc. New Zealand Grassl. Assoc. 74, 153–158. doi: 10.33584/jnzg.2012.74.2861
Jaklitsch, W. M., and Voglmayr, H. (2015). Biodiversity of Trichoderma (Hypocreaceae) in Southern Europe and Macaronesia. Stud. Mycol. 80, 1–87. doi: 10.1016/j.simyco.2014.11.001
Jamieson, M. A., Trowbridge, A. M., Raffa, K. F., and Lindroth, R. L. (2012). Consequences of climate warming and altered precipitation patterns for plant-insect and multitrophic interactions. Plant Physiol. 160, 1719–1727. doi: 10.1104/pp.112.206524
Johnson, L. J., de Bonth, A. C. M., Briggs, L. R., Caradus, J. R., Finch, S. C., Fleetwood, D. J., et al. (2013). The exploitation of epichloae endophytes for agricultural benefit. Fungal Diversity 60, 171–188. doi: 10.1007/s13225-013-0239-4
Johnson, S. N., and McNicol, J. W. (2010). Elevated CO2 and aboveground belowground herbivory by the clover root weevil. Oecologia. 162, 209–216. doi: 10.1007/s00442-009-1428-4
Julier, B., Bournoville, R., Landre, B., Ecalle, C., and Carre, S. (2004). Genetic analysis of lucerne (Medicago sativa L.) seedling resistance to pea aphid (Acyrthosiphum pisum Harris). Euphytica. 138, 133–139. doi: 10.1023/B:EUPH.0000046794.64020.00
Kain, W. M., and Biggs, D. R. (1980). Effect of pea aphid and bluegreen lucerne aphid (Acyrthosiphon spp.) on coumestrol levels in herbage of lucerne (Medicago sativa). New Zealand J. Agric. Res. 23, 563–568. doi: 10.1080/00288233.1980.10417883
Kain, W. M., and Trought, T. E. T. (1982). “Insect pests of lucerne in New Zealand.” In: Lucerne for the 80's: Agronomy Society of New Zealand special publication No. 1, Wynn-Williams, R. B., (eds) p. 49–59. Christchurch, NZ: Agronomy Society of New Zealand.
Kang, W., Xu, L., Jiang, Z., and Shi, S. (2019). Genetic diversity and symbiotic efficiency difference of endophytic rhizobia of Medicago sativa. Can. J. Microbiol. 65, 68-83. doi: 10.1139/cjm-2018-0158
Kaul, S., Sharma, T., and Dhar, M. K. (2016). “Omics” tools for better understanding the plant-endophyte interactions. Front. Plant Sci. 7, 955–955. doi: 10.3389/fpls.2016.00955
Ke, J., Wang, B., and Yoshikuni, Y. (2021). Microbiome engineering: synthetic biology of plant-associated microbiomes in sustainable agriculture. Trends Biotech. 39(3), 244-261. doi: 10.1016/j.tibtech.2020.07.008
Kearl, J., McNary, C., Lowman, J. S., Mei, C., Aanderud, Z. T., Smith, S. T., et al. (2019). Salt-tolerant halophyte rhizosphere bacteria stimulate growth of alfalfa in salty soil. Front. Microbiol. 10, 1849. doi: 10.3389/fmicb.2019.01849
Khalifa, A. Y., Alsyeeh, A. M., Almalki, M. A., and Saleh, F. A. (2016). Characterization of the plant growth promoting bacterium, Enterobacter cloacae MSR1, isolated from roots of non-nodulating Medicago sativa. Saudi J. Biol. Sci.s 23, 79-86. doi: 10.1016/j.sjbs.2015.06.008
Khare, E., Mishra, J., and Arora, N. K. (2018). Multifaceted interactions between endophytes and plant: developments and prospects. Front. Microbiol. 9, 2732-2732. doi: 10.3389/fmicb.2018.02732
Kubicek, C. P., Bissett, J., Druzhinina, I., Kullnig-Gradinger, C., and Szakacs, G. (2003). Genetic and metabolic diversity of Trichoderma: a case study on South-East Asian isolates. Fungal Genet. Biol. 38, 310-319. doi: 10.1016/S1087-1845(02)00583-2
Kumar, V., Almomin, S., Al-Aqeel, H., Al-Salameen, F., Nair, S., and Shajan, A. (2018). Metagenomic analysis of rhizosphere microflora of oil-contaminated soil planted with barley and alfalfa. PloS ONE. 13, e0202127–e0202127. doi: 10.1371/journal.pone.0202127
Labandeira, C. C. (2013). A paleobiologic perspective on plant-insect interactions. Curr. Opin. Plant Biol. 16, 414-421. doi: 10.1016/j.pbi.2013.06.003
Lacey, L. A., Grzywacz, D., Shapiro-Ilan, D. I., Frutos, R., Brownbridge, M., and Goettel, M. S. (2015). Insect pathogens as biological control agents: back to the future. J. Invertebr. Pathol. 132:1-41. doi: 10.1016/j.jip.2015.07.009
Lamb, J. F. S., Sheaffer, C. C., Rhodes, L. H., Sulc, R. M., Undersander, D. J., and Brummer, E. C. (2006). Five decades of alfalfa cultivar improvement: impact on forage yield, persistence, and nutritive value. Crop Sci. 46:902–909. doi: 10.2135/cropsci2005.08-0236
Le Cocq, K., Gurr, S. J., Hirsch, P. R., and Mauchline, T. H. (2017). Exploitation of endophytes for sustainable agricultural intensification. Mol. Plant. Pathol. 18, 469–473. doi: 10.1111/mpp.12483
Leath, K. T., Erwin, D. C., and Griffin, G. D. (1988). “Diseases and nematodes”, in Alfalfa and Alfalfa Improvement-Agronomy Monograph no. 29, Hanson, A. A., Barnes, D. K., Hill, R. R. Jr. Madison, WI: ASA-CSSA-SSSA 621–670. doi: 10.2134/agronmonogr29.c21
Lee, S. H., Yun, S. H., Chun, J., and Kim, D. H. (2017). Characterization of a novel dsRNA mycovirus of Trichoderma atroviride NFCF028. Arch. Virol. 162, 1073–1077. doi: 10.1007/s00705-016-3214-z
Lemaire, G., Giroud, B., Bathily, B., Lecomte, P., and Corniaux, C. (2019). “Chapter 17 - Toward integrated crop-livestock systems in West Africa: A project for dairy production along Senegal River”, in Agroecosystem diversity: Reconciling contemporary agriculture and environmental quality, Lemaire G., Carvalho, P.C.D.F., Kronberg, S., Recous, S., (eds). London. UK: Academic Press. p. 275–285. doi: 10.1016/B978-0-12-811050-8.00017-0
Lemoine, M. M., Engl, T., and Kaltenpoth, M. (2020). Microbial symbionts expanding or constraining abiotic niche space in insects. Curr. Opin. Insect Sci. 39, 14-20. doi: 10.1016/j.cois.2020.01.003
Lewis, L. C., and Cossentine, J. E. (1986). Season long intraplant epizootics of entomopathogens, Beauveria bassiana and Nosema pyrausta, in a corn agroecosystem. Entomophaga. 31, 363–369. doi: 10.1007/BF02373152
Lewis, W. J., Van Lenteren, J. C., Phatak, S. C., and Tumlinson, I. I. I. J. H. (1997). A total system approach to sustainable pest management. Proc. Natl. Acad. Sci. U.S.A. 94, 12243–12248. doi: 10.1073/pnas.94.23.12243
Li, C., Sun, Q., Gou, Y., Zhang, K., Zhang, Q., Zhou, J.-J., et al. (2021). Long-Term Effect of Elevated CO2 on the development and nutrition contents of the pea aphid (Acyrthosiphon pisum). Front. Physiol. 12, 688220. doi: 10.3389/fphys.2021.688220
Li, G. D., Nie, Z. N., Boschma, S. P., Dear, B. S., Lodge, G. M., Hayes, R. C., et al. (2010). Persistence and productivity of Medicago sativa subspecies sativa, caerulea, falcata and varia accessions at three intermittently dry sites in south-eastern Australia. Crop Pasture Sci. 61, 645–658. doi: 10.1071/CP09360
Li, J., Gu, H.-C., Liu, Y., Wei, S.-H., Hu, G., Wang, X.-M., et al. (2021). RNA-seq reveals plant virus composition and diversity in alfalfa, thrips, and aphids in Beijing, China. Arch. Virol. 166, 1711–1722. doi: 10.1007/s00705-021-05067-1
Li, S., Du, G., Yin, X., Liu, X., Jiang, X., Li, Y., et al. (2020). Research advance in forage diseases, insect pests and rodents in China. Chinese J. Biol. Cont. 36, 9–16. doi: 10.16409/j.cnki.2095-039x.2020.01.002
Li, Y., Nan, Z., and Duan, T. (2019). Rhizophagus intraradices promotes alfalfa (Medicago sativa) defense against pea aphids (Acyrthosiphon pisum) revealed by RNA-Seq analysis. Mycorrhiza. 29, 623–635. doi: 10.1007/s00572-019-00915-0
Liang, J., Xia, J., Liu, L., and Wan, S. (2013). Global patterns of the responses of leaf-level photosynthesis and respiration in terrestrial plants to experimental warming. J. Plant Ecol. 6, 437–447. doi: 10.1093/jpe/rtt003
Liotti, R. G., Da Silva Figueiredo, M. I., Da Silva, G. F., De Mendonça, E. A. F., and Soares, M. A. (2018). Diversity of cultivable bacterial endophytes in Paullinia cupana and their potential for plant growth promotion and phytopathogen control. Microbiol. Res. 207, 8–18. doi: 10.1016/j.micres.2017.10.011
Liu, W.-X., Zhang, Z.-S., Chen, S.-Y., Ma, L.-C., Wang, H.-C., Dong, R., et al. (2016). Global transcriptome profiling analysis reveals insight into saliva-responsive genes in alfalfa. Plant Cell Rep. 35, 561–571. doi: 10.1007/s00299-015-1903-9
Lodewyckx, C., Vangronsveld, J., Porteous, F., Moore, E. R. B., Taghavi, S., Mezgeay, M., et al. (2002). Endophytic bacteria and their potential applications. Crit. Rev. Plant Sci. 21, 583–606. doi: 10.1080/0735-260291044377
Lopes, R., Tsui, S., Gonçalves, P., and De Queiroz, M. V. (2018). A look into a multifunctional toolbox: endophytic Bacillus species provide broad and underexploited benefits for plants. World J. Microbiol. Biotechnol. 34, 94. doi: 10.1007/s11274-018-2479-7
López, J. L., Alvarez, F., Príncipe, A., Salas, M. E., Lozano, M. J., Draghi, W. O., et al. (2018). Isolation, taxonomic analysis, and phenotypic characterization of bacterial endophytes present in alfalfa (Medicago sativa) seeds. J. Biotechnol. 267, 55–62. doi: 10.1016/j.jbiotec.2017.12.020
Lovinger, A., Liewehr, D., and Lamp, W. O. (2000). Glandular trichomes on alfalfa impede searching behavior of the potato leafhopper parasitoid. Biol. Control. 18, 187–192. doi: 10.1006/bcon.2000.0835
Maleck, K., and Dietrich, R. A. (1999). Defense on multiple fronts: How do plants cope with diverse enemies? Trends Plant Sci. 4, 215-219. doi: 10.1016/S1360-1385(99)01415-6
Manglitz, G. R., and Ratcliffe, R. H. (1988). “Insects and Mites.” in Alfalfa and Alfalfa Improvement-Agronomy Monograph no. 29. Hanson, A. A., Barnes, D. K., Hill R. R., Jr., (eds). Madison, WI: ASA-CSSA-SSSA. p. 671–704. doi: 10.2134/agronmonogr29.c22
Mauch-Mani, B., Baccelli, I., Luna, E., and Flors, V. (2017). Defense priming: an adaptive part of induced resistance. Annu. Rev. of Plant Biol. 68, 485–512. doi: 10.1146/annurev-arplant-042916-041132
McNeill, M. R., Tu, X., Ferguson, C. M., Ban, L., Hardwick, S., Zhang, R., et al. (2021). Diversity and impacts of key grassland and forage arthropod pests in China and New Zealand: A review of IPM and biosecurity initiatives. Neobiota. 65, 137–168. doi: 10.3897/neobiota.65.61991
Mehrparvar, M., Rajaei, A., Rokni, M., Balog, A., and Loxdale, H. D. (2019). 'Bottom-up' effects in a tritrophic plant-aphid-parasitoid system: why being the perfect host can have its disadvantages. Bull. Entomol. Res. 109, 831–839. doi: 10.1017/S0007485319000129
Meiners, T. (2015). Chemical ecology and evolution of plant-insect interactions: a multitrophic perspective. Curr. Opinion Insect Sci. 8, 22–28. doi: 10.1016/j.cois.2015.02.003
Michaud, J. P., Pell, J. K., and Vega, F. E. (2018). When insect endosymbionts and plant endophytes mediate biological control outcomes. Biol. Control 116, 1–2. doi: 10.1016/j.biocontrol.2017.11.003
Michaud, R., Lehman, W. F., and Rumbaugh, M. D. (1988). “World distribution and historical development,” in Alfalfa and Alfalfa Improvement. Agronomy Monograph no. 29, Hanson, A. A., Barnes, D. K., Hill R. R., Jr., (eds). Maddison, WI: ASA-CSSA-SSSA. p. 25–92. doi: 10.2134/agronmonogr29.c2
Moot, D. J. (2012). An overview of dryland legume research in New Zealand. Crop and Pasture Sci. 63, 726–733. doi: 10.1071/CP12103
Muresu, R., Polone, E., Sulas, L., Baldan, B., Tondello, A., Delogu, G., et al. (2008). Coexistence of predominantly nonculturable rhizobia with diverse, endophytic bacterial taxa within nodules of wild legumes. FEMS Microbiol. Ecol. 63, 383–400. doi: 10.1111/j.1574-6941.2007.00424.x
National Animal Husbandry Service. (2017). Forage and Grass Statistics of China. Beijing: China Agricultural Press. p. 62–63.
Nawrocka, J., and Ma.olepsza, U. (2013). Diversity in plant systemic resistance induced by Trichoderma. Biol. Control. 67, 149–156. doi: 10.1016/j.biocontrol.2013.07.005
Nerva, L., Varese, G. C., Falk, B. W., and Turina, M. (2017). Mycoviruses of an endophytic fungus can replicate in plant cells: Evolutionary implications. Sci. Rep. 7, 1908. doi: 10.1038/s41598-017-02017-3
Noori, F., Etesami, H., Najafi Zarini, H., Khoshkholgh-Sima, N. A., Hosseini Salekdeh, G., and Alishahi, F. (2018). Mining alfalfa (Medicago sativa L.) nodules for salinity tolerant non-rhizobial bacteria to improve growth of alfalfa under salinity stress. Ecotoxicol. Environ. Saf. 162, 129–138. doi: 10.1016/j.ecoenv.2018.06.092
Nordkvist, M., Klapwijk, M. J., Edenius, L., Gershenzon, J., Schmidt, A., and Björkman, C. (2019). Trait-mediated indirect interactions: Moose browsing increases sawfly fecundity through plant-induced responses. Ecol. Evol. 9, 10615–10629. doi: 10.1002/ece3.5581
O'Hara, G. W. (2001). Nutritional constraints on root nodule bacteria affecting symbiotic nitrogen fixation: a review. Aust. J. Exp. Agric. 41, 417–433. doi: 10.1071/EA00087
Oliver, K. M., and Martinez, A. J. (2014). How resident microbes modulate ecologically-important traits of insects. Curr. Opinion Insect Sci. 4, 1–7. doi: 10.1016/j.cois.2014.08.001
Ormskirk, M. M., Narciso, J., Hampton, J. G., and Glare, T. R. (2019). Endophytic ability of the insecticidal bacterium Brevibacillus laterosporus in Brassica. PLoS ONE. 14, e0216341. doi: 10.1371/journal.pone.0216341
Pan, Q., Shikano, I., Hoover, K., Liu, T. X., and Felton, G. W. (2019). Pathogen-mediated tritrophic interactions: Baculovirus-challenged caterpillars induce higher plant defenses than healthy caterpillars. J. Chem. Ecol. 45, 515–524. doi: 10.1007/s10886-019-01077-1
Pedrono, M., Locatelli, B., Ezzine-de-Blas, D., Pesche, D., Morand, S., and Binot, A. (2016). “Impact of climate change on ecosystem services”, in Climate Change and Agriculture Worldwide, Torquebiau, E., (eds). Dordrecht: Springer. doi: 10.1007/978-94-017-7462-8_19
Pembleton, K. G., Smith, R. S., Rawnsley, R. P., Donaghy, D. J., and Humphries, A. W. (2010). Genotype by environment interactions of lucerne (Medicago sativa L.) in a cool temperate climate. Crop Pasture Sci. 61, 493–502. doi: 10.1071/CP09269
Pickett, J. A., and Khan, Z. R. (2016). Plant volatile-mediated signalling and its application in agriculture: successes and challenges. New Phytol. 212, 856–870. doi: 10.1111/nph.14274
Pickett, J. A., Woodcock, C. M., Midega, C. O., and Khan, Z. R. (2014). Push-pull farming systems. Curr. Opin. Biotechnol. 26, 125–132. doi: 10.1016/j.copbio.2013.12.006
Pineda, A., Kaplan, I., Hannula, S. E., Ghanem, W., and Bezemer, T. M. (2019). Conditioning the soil microbiome through plant–soil feedbacks suppresses an aboveground insect pest. New Phytol. 226, 595-608. doi: 10.1111/nph.16385
Pinski, A., Betekhtin, A., Hupert-Kocurek, K., Mur, L.,a,.J, and Hasterok, R. (2019). Defining the genetic basis of plant?endophytic bacteria interactions. Int. J. Mol. Sci. 20(8), 1947, doi: 10.3390/ijms20081947
Pixley, K. V., Falck-Zepeda, J. B., Giller, K. E., Glenna, L. L., Gould, F., Mallory-Smith, C. A., et al. (2019). Genome editing, gene drives, and synthetic biology: will they contribute to disease-resistant crops, and who will benefit? Annu. Rev. Phytopathol. 57, 165–188. doi: 10.1146/annurev-phyto-080417-045954
Purves, R. G., Hood, N. D., and Dunbier, M. W. (1981). The effect of cutting management and fungicide application on coumestrol levels in three lucerne cultivars. Proc. New Zealand Weed Pest Cont. Conf. 34, 25–28. doi: 10.30843/nzpp.1981.34.10622
Quispel, A. (1992). “A search of signal in endophytic microorganisms,” in Molecular Signals in Plant-Microbe Communications, Verma, D.P.S. Boca Raton, FL: CRS Press. p. 475–491.
Raffa, K. F., Bonello, P., and Orrock, J. L. (2020). Why do entomologists and plant pathologists approach trophic relationships so differently? Identifying biological distinctions to foster synthesis. New Phytol. 225, 609–620. doi: 10.1111/nph.16181
Rasmann, S., Köllner, T. G., Degenhardt, J., Hiltpold, I., Toepfer, S., Kuhlmann, U., et al. (2005). Recruitment of entomopathogenic nematodes by insect-damaged maize roots. Nature 434, 732–737. doi: 10.1038/nature03451
Rasmussen, S., Parsons, A. J., and Jones, C. S. (2012). Metabolomics of forage plants: A review. Ann. Bot. 110, 1281–1290. doi: 10.1093/aob/mcs023
Ratcliffe, R. H., and Campbell, T. A. (1995). Selection within wild Turkish alfalfa germplasm for alfalfa weevil (Coleoptera: Curculionidae) resistance. J. Entomol. Sc. 30, 120–129. doi: 10.18474/0749-8004-30.1.120
Ricciardi, A., Iacarella, J. C., Aldridge, D. C., Blackburn, T. M., Carlton, J. T., Catford, J. A., et al. (2021). Four priority areas to advance invasion Sci. in the face of rapid environmental change. Environ. Rev. 29, 119–141. doi: 10.1139/er-2020-0088
Rodriguez-Llorente, I., Caviedes, M. A., Dary, M., Palomares, A. J., Cánovas, F. M., and Peregrín-Alvarez, J. M. (2009). The symbiosis interactome: A computational approach reveals novel components, functional interactions and modules in Sinorhizobium meliloti. BMC Syst. Biol. 3, 63–63. doi: 10.1186/1752-0509-3-63
Roumagnac, P., Granier, M., Bernardo, P., Deshoux, M., Ferdinand, R., Galzi, S., et al. (2015). Alfalfa leaf curl virus: An aphid-transmitted geminivirus. J. Virology. 89, 9683–9688. doi: 10.1128/JVI.00453-15
Ruckle, M., Meier, M., Frey, L., Eicke, S., Kölliker, R., Zeeman, S., et al. (2017). Diurnal leaf starch content: An orphan trait in forage legumes. Agronomy. 7, 16, doi: 10.3390/agronomy7010016
Ruiu, L. (2013). Brevibacillus laterosporus, a pathogen of invertebrates and a broad-spectrum antimicrobial species. Insects 4(3), 476–492. doi: 10.3390/insects4030476
Saidi, S., Cherif-Silini, H., Chenari Bouket, A., Silini, A., Eshelli, M., Luptakova, L., et al. (2021). Improvement of Medicago sativa crops productivity by the co-inoculation of Sinorhizobium meliloti–actinobacteria under salt stress. Curr. Microbiol. 78, 1344–1357. doi: 10.1007/s00284-021-02394-z
Sanzari, I., Leone, A., and Ambrosone, A. (2019). Nanotechnology in plant science: to make a long story short. Front. Bioeng. Biotechnol. 7, 120. doi: 10.3389/fbioe.2019.00120
Savi, D. C., Aluizio, R., and Glienke, C. (2019). Brazilian plants: an unexplored source of endophytes as producers of active metabolites. Planta Medica 85, 619–636. doi: 10.1055/a-0847-1532
Scasta, J. D., Trostle, C. L., and Foster, M. A. (2012). Evaluating alfalfa (Medicago sativa L.) cultivars for salt tolerance using laboratory, greenhouse and field methods. J. Agric. Sci. 4, 90–100. doi: 10.5539/jas.v4n6p90
Schaedel, M., Hidrobo, G., and Grossman, J. (2021). From microns to meters: Exploring advances in legume microbiome diversity for agroecosystem benefits. Front. Sustain. Food Syst. 5. doi: 10.3389/fsufs.2021.668195
Schardl, C. L., Florea, S., Pan, J., Nagabhyru, P., Bec, S., and Calie, P. J. (2013). The Epichloae: alkaloid diversity and roles in symbiosis with grasses. Curr. Opin. Plant Biol. 16, 480–488. doi: 10.1016/j.pbi.2013.06.012
Schierstaedt, J., Bziuk, N., Kuzmanovi,ć, N., Blau, K., Smalla, K., and Jechalke, S. (2019). Role of plasmids in plant-bacteria interactions. Curr Issues Mol Biol. 30, 17–38. doi: 10.21775/cimb.030.017
Sewell, J. C., Hill, R. D., and Reich, J. (2011). Persistence of grazing tolerant lucernes under Australian conditions. Pasture Persistence Symposium. Grassl. Res. Pract. Ser. 15, 187–190. doi: 10.33584/rps.15.2011.3201
Shi, S., Nan, L., and Smith, K. F. (2017). The current status, problems, and prospects of alfalfa (Medicago sativa L.) breeding in China. Agronomy. 7, 1–11. doi: 10.3390/agronomy7010001
Shikano, I., Rosa, C., Tan, C. W., and Felton, G. W. (2017). Tritrophic interactions: Microbe-mediated plant effects on insect herbivores. Annu. Rev. Phytopathol. 55, 313–331. doi: 10.1146/annurev-phyto-080516-035319
Small, E. (1996). Adaptions to herbivory in alfalfa (Medicago sativa). Can. J. Bot. 74, 807–822. doi: 10.1139/b96-102
Smith, A. H., OfConnor, M. P., Deal, B., Kotzer, C., Lee, A., Wagner, B., et al. (2021). Does getting defensive get you anywhere?. Seasonal balancing selection, temperature, and parasitoids shape real world, protective endosymbiont dynamics in the pea aphid. Mol. Ecol. 30, 2449–2472. doi: 10.1111/mec.15906
Smith, G. (2015). The strategic use of lucerne (Medicago sativa) on irrigated dairy farms. PhD Thesis. Lincoln University, New Zealand.
Soares, J. C., Santos, C. S., Carvalho, S. M. P., Pintado, M. M., and Vasconcelos, M. W. (2019). Preserving the nutritional quality of crop plants under a changing climate: importance and strategies. Plant Soil. 443, 1–26. doi: 10.1007/s11104-019-04229-0
Sochard, C., Leclair, M., Simon, J. C., and Outreman, Y. (2019). Host plant effects on the outcomes of defensive symbioses in the pea aphid complex. Evol. Ecol. 33, 651–669. doi: 10.1007/s10682-019-10005-4
Stajković, O., De Meyer, S., Miličić, B., Willems, A., and Delić, D. (2009). Isolation and characterization of endophytic non-rhizobial bacteria from root nodules of alfalfa (Medicago sativa L.). Botanica Serbica 33, 107–114.
Strobel, G. (2018). The emergence of endophytic microbes and their biological promise. J. Fungi 4, 57, doi: 10.3390/jof4020057
Summers, C. G. (1998). Integrated pest management in forage alfalfa. Integrated Pest Manag. Rev. 3, 127–154. doi: 10.1023/A:1009654901994
Sveen, T. R., Netherway, T., Juhanson, J., Oja, J., Borgström, P., Viketoft, M., et al. (2021). Plant-microbe interactions in response to grassland herbivory and nitrogen eutrophication. Soil Biol. Biochem. 156, 108208. doi: 10.1016/j.soilbio.2021.108208
Thomson, L. J., Macfadyen, S., and Hoffmann, A. A. (2010). Predicting the effects of climate change on natural enemies of agricultural pests. Biol. Control. 52, 296–306. doi: 10.1016/j.biocontrol.2009.01.022
Tougeron, K., Brodeur, J., Le Lann, C., and van Baaren, J. (2020). How climate change affects the seasonal ecology of insect parasitoids. Ecol. Entomol. 45, 167–181. doi: 10.1111/een.12792
Trebicki, P., Dáder, B., Vassiliadis, S., and Fereres, A. (2017). Insect–plant–pathogen interactions as shaped by future climate: effects on biology, distribution, and implications for agriculture. Insect Sci. 24, 975–989. doi: 10.1111/1744-7917.12531
Trumble, J. T., Kolodny-Hirsch, D. M., and Ting, I. P. (1993). Plant compensation for arthropod herbivory. Annu. Rev. Entomol. 38, 93–119. doi: 10.1146/annurev.en.38.010193.000521
Turlings, T. C. J., and Erb, M. (2018). Tritrophic interactions mediated by herbivore-induced plant volatiles: Mechanisms, ecological relevance, and application potential. Annu. Rev. Entomol. 63, 433–452. doi: 10.1146/annurev-ento-020117-043507
Ulloa-Muñoz, R., Olivera-Gonzales, P., Castañeda-Barreto, A., Villena, G. K., and Tamariz-Angeles, C. (2020). Diversity of endophytic plant-growth microorganisms from Gentianella weberbaueri and Valeriana pycnantha, highland Peruvian medicinal plants. Microbiol. Res. 233, 126413. doi: 10.1016/j.micres.2020.126413
Undersander, D., Cosgrove, D., Cullen, E., Grau, C., Rice, M. E., Renz, M., et al. (2011). Alfalfa management guide. Maddison, WI: ASA-CSSA-SSSA. doi: 10.2134/2011.alfalfamanagementguide
van Zijll de Jong, E., Roush, T. L., Glare, T. R., and Hampton, J. G. (2016). Discovery of two Brevibacillus laterosporus isolates from Brassica with insecticidal properties against diamondback moth. Biocontrol Sci. Technol. 26, 426–431. doi: 10.1080/09583157.2015.1118437
Vance, C. P., Heichel, G. H., Barnes, D. K., Bryan, J. W., and Johnson, L. E. (1979). Nitrogen fixation, nodule development, and vegetative regrowth of alfalfa (Medicago sativa L.) following harvest. Plant Physiol. 64, 1–8. doi: 10.1104/pp.64.1.1
Venkatesh, J., and Kang, B. C. (2019). Current views on temperature-modulated R gene-mediated plant defense responses and tradeoffs between plant growth and immunity. Curr. Opin. Plant Biol. 50, 9–17. doi: 10.1016/j.pbi.2019.02.002
Veronesi, F., Brummer, E. C., and Huyghe, C. (2010). “Alfalfa,” in Fodder Crops and Amenity Grasses. Handbook of Plant Breeding, Boller, B., Posselt, U.K., and Veronesi, F. New York: Springer. 395–437 doi: 10.1007/978-1-4419-0760-8_17
Wang, X., Gao, Y., Chen, Z., Li, J., Huang, J., Cao, J., et al. (2019). (E)-β-farnesene synthase gene affects aphid behavior in transgenic Medicago sativa. Pest Manag. Sci. 75, 622-631. doi: 10.1002/ps.5153
Wang, X., Zhang, J., Liu, C., Wang, R.-Q., Tan, Z., Wang, Y.-H., et al. (2021). Investigating incidence and distribution of alfalfa-infecting viruses in China. Agric. Res. Tech Open Access J. 25, 556314.
Ward, S. F., Aukema, B. H., Fei, S., and Liebhold, A. M. (2020). Warm temperatures increase population growth of a nonnative defoliator and inhibit demographic responses by parasitoids. Ecology. 101, e03156. doi: 10.1002/ecy.3156
Wemheuer, F., Wemheuer, B., Kretzschmar, D., Pfeiffer, B., Herzog, S., Daniel, R., et al. (2016). Impact of grassland management regimes on bacterial endophyte diversity differs with grass species. Lett. Appl. Microbiol. 62, 323–329. doi: 10.1111/lam.12551
Whitaker, B. K., and Bakker, M. G. (2019). Bacterial endophyte antagonism toward a fungal pathogen in vitro does not predict protection in live plant tissue. FEMS Microbiol. Ecol. 95, fiy237. doi: 10.1093/femsec/fiy237
Whitney, H. M., and Federle, W. (2013). Biomechanics of plant-insect interactions. Curr. Opin. Plant Biol. 16, 105–111. doi: 10.1016/j.pbi.2012.11.008
Whittaker, J. B. (1999). Impacts and responses at population level of herbivorous insects to elevated CO2. Eur. J. Entomol. 96, 149–156.
Wu, B., Li, M., Liu, C., and Jiang, X. (2020). The insight of mycovirus from Trichoderma spp. Agric. Res. Tech. Open Access. J. 24, 38–41.
Wu, F., Shi, S., Li, Y.-Z., Miao, J.-M., Kang, W.-J., Zhang, J., et al. (2021). Physiological and biochemical response of different resistant alfalfa cultivars against thrips damage. Physiol. Mol. Bio.l Plants 27, 649–663. doi: 10.1007/s12298-021-00961-z
Wu, Z.-G., Zhu, M., Zhang, R., Zhang, Z., and Li, Z. (2013). An assessment of economic losses of alfalfa caused by Therioaphis trifolii (Homoptera: Callaphididae) in China. Ningxia J. Agric. Forestry Sci. Technol. 54, 74–77.
Xu, R., Zhao, H., Liu, G., You, Y.-L., Ma, L., Liu, N., et al. (2021). Effects of nitrogen and maize plant density on forage yield and nitrogen uptake in an alfalfa–silage maize relay intercropping system in the North China Plain. Field Crops Res. 263, 108068. doi: 10.1016/j.fcr.2021.108068
Yang, L. H., Postema, E. G., Hayes, T. E., Lippey, M. K., and MacArthur-Waltz, D. J. (2021). The complexity of global change and its effects on insects. Curr. Opin. Insect Sci. 47, 90–102, doi: 10.1016/j.cois.2021.05.001
Yuegao, H., Cash, D., Kechang, L., Suqin, W., Ping, Z., and Rong, G. (2009). “Global status and development trends of alfalfa”, in Alfalfa management guide for Ningxia. p. 1–14.
Zakhia, F., Jeder, H., Domergue, O., Willems, A., Cleyet-Marel, J. C., Gillis, M., et al. (2004). Characterisation of wild legume nodulating bacteria (LNB) in the infra-arid zone of Tunisia. Syst. Appl. Microbiol. 27, 380–395. doi: 10.1078/0723-2020-00273
Zhang, F., Xu, X., Huo, Y., and Xiao, Y. (2019). Trichoderma-inoculation and mowing synergistically altered soil available nutrients, rhizosphere chemical compounds and soil microbial community, potentially driving alfalfa growth. Front. Microbiol. 9, 3241. doi: 10.3389/fmicb.2018.03241
Zhang, R., Xian, C., Yang, F., Ma, J., and Zhang, S. (2005a). Studies on the yield damage and economic threshold of Loxostege sticticalis and Loxostege verticalis to Medicago sativa. Acta Prataculturae Sinica 14, 121–123.
Zhang, R., Yang, F., Xian, C., Ma, J., and Zhang, S. H. (2005b). A study on the yield loss and economic threshold of alfalfa damaged by thrip, Odentothrips lati. Plant Prot. 31, 47–49.
Zhang, W., Hou, L., Yang, J., Song, S., Mao, X., Zhang, Q., et al. (2018). Establishment and management of alfalfa pasture in cold regions of China. Chinese Sci. Bull. 63, 1651–1663. doi: 10.1360/N972017-01181
Zhang, X., Pen, R., and Hu, G. (2017). Effect of Odontothrips loti nymphs sustainable damage on alfalfa growth. Grassland and Turf 37, 8–13.
Zhang, Y.-L., and Li, X. (2019). Salicylic acid: iosynthesis, perception, and contributions to plant immunity. Curr. Opin. Plant Biol. 50, 29-36. doi: 10.1016/j.pbi.2019.02.004
Zogli, P., Pingault, L., Grover, S., and Louis, J. (2020). Ento(o)mics: the intersection of ‘omic’ approaches to decipher plant defense against sap-sucking insect pests. Curr. Opin. Plant Biol. 56, 153–161. doi: 10.1016/j.pbi.2020.06.002
Zydenbos, S., Barratt, B., Bell, N., Ferguson, C., Gerard, P., McNeill, M., et al. (2011). “The impact of invertebrate pests on pasture persistence and their interrelationship with biotic and abiotic factors,” in Pasture Persistence - Grassland Research and Practice Series 15, Mercer, C. F. Dunedin: New Zealand Grassland Association. 109–118. doi: 10.33584/rps.15.2011.3203
Keywords: alfalfa, insect pests, multitrophic interactions, biocontrol, plant resistance mechanisms, endophytes, rhizosphere, climate–change
Citation: McNeill MR, Tu X, Altermann E, Beilei W and Shi S (2022) Sustainable Management of Medicago sativa for Future Climates: Insect Pests, Endophytes and Multitrophic Interactions in a Complex Environment. Front. Agron. 4:825087. doi: 10.3389/fagro.2022.825087
Received: 30 November 2021; Accepted: 15 March 2022;
Published: 26 April 2022.
Edited by:
Michael J. Stout, Louisiana State University, United StatesReviewed by:
Sophie Trouvelot, Université de Bourgogne, FranceAyman Mostafa, University of Arizona, United States
Copyright © 2022 McNeill, Tu, Altermann, Beilei and Shi. This is an open-access article distributed under the terms of the Creative Commons Attribution License (CC BY). The use, distribution or reproduction in other forums is permitted, provided the original author(s) and the copyright owner(s) are credited and that the original publication in this journal is cited, in accordance with accepted academic practice. No use, distribution or reproduction is permitted which does not comply with these terms.
*Correspondence: Mark R. McNeill, mark.mcneill@agresearch.co.nz
†ORCID: Mark R. McNeill orcid.org/0000-0001-9140-610X
Eric Altermann orcid.org/0000-0003-1376-1549
Shengjing Shi orcid.org/0000-0002-8938-1932